Molecular-structure-NAD.jpg
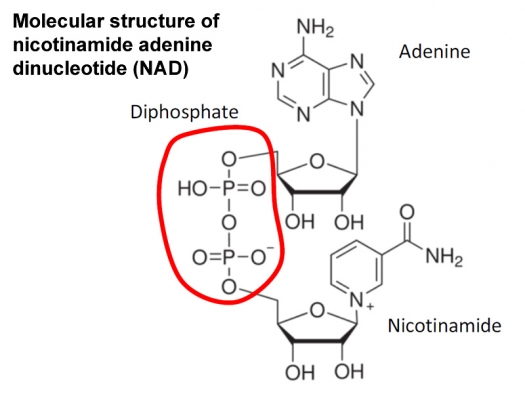
Stephanie Seneff
Figure 1: Molecular structure of nicotinamide adenine dinucleotide (NAD). Nicotinamide and adenine are the nucleotides. Note the two phosphates circled in the middle.
1 Introduction
For the past eight years, I have been studying glyphosate, the active ingredient in the pervasive herbicide, Roundup, and it has taken me this long just to begin to understand the full ramifications of its insidious, cumulative toxic effects. I have observed a worldwide trend towards upticks in diabetes and obesity once fast food from the West becomes widely available in a given country. Typically, autism rates start going up as well, and then Alzheimer's starts showing up more prevalently in younger and younger populations, as we are now observing in the United States and other Western countries, where glyphosate has contaminated the food supply for well over two decades.
Glyphosate kills all plants except those that are engineered through GMO technology to resist it. Its main toxic effect to plants is its disruption of the so-called shikimate biological pathway, a pathway that is essential in plants (and in many microbes) but which human cells don't use at all. This is the main explanation for why it is claimed to be nearly nontoxic to humans. The fact is that its acute toxic effects (very short term) on humans are minimal. What I believe it does that is so insidious and dangerous is that it gets into proteins by mistake in place of the coding amino acid glycine, and, for some proteins, this totally disrupts their ability to do their job. It can even cause the build-up of amyloid beta plaque associated with Alzheimer's disease1.
Glyphosate is a pretty simple molecule. It is a complete clone of glycine, except that it has swapped out a hydrogen atom and replaced it with something called a “methylphosphonyl group” (for you chemistry buffs, a methyl is CH3 and phosphonyl is PO(OH)2). The idea that glyphosate substitutes for glycine during protein synthesis was something that was proposed to me by Anthony Samsel in December of 2015. I had thought of this possibility but rejected it, because I mistakenly thought the extra methylphosphonyl group would prevent it from hooking up into the amino acid chain. However, once he suggested the idea, I took a closer look, and I realized that there is a coding amino acid called proline that, like glyphosate, attaches to two carbon atoms rather than only one. The extra material attached to the nitrogen atom in proline doesn't prevent it from hooking up to the carboxyl group (COOH) of the adjacent amino acid in the chain. So maybe glyphosate could, too?
Clearly glyphosate can't fit everywhere that glycine can fit. If the neighboring amino acids are big and bulky, glyphosate's extra methylphosphonate will have nowhere to go.
However, one place where it is likely to fit well is in a protein that binds phosphate at the site where phosphate binds. The methylphosphonate unit can occupy the roomy hole where phosphate in the substrate that binds to the enzyme is supposed to fit. This of course totally prevents the substrate from binding, thus ruining the enzyme's ability to carry out the reaction it normally achieves2.
This is exactly what I think happens when glyphosate suppresses the activity of 5- enolpyruvylshikimate-3-phosphate (EPSP) synthase, the enzyme that it blocks in the shikimate pathway to kill plants. EPSP synthase binds phosphoenolpyruvate (PEP) at a site where there is a highly conserved glycine residue at the place where phosphate binds. Researchers have consistently found that swapping out that glycine for the amino acid alanine (through a mutation in the genetic code for the protein) results in a version of the protein that is completely insensitive to glyphosate's effects3. In fact, they have used this idea to create glyphosate resistance in genetically modified crops. The simplest way to explain glyphosate's effects is to propose that it displaces glycine in the enzyme, and its bulky methylphosphonate group blocks binding to PEP.
The shikimate pathway produces the three aromatic amino acids, tryptophan, tyrosine, and phenylalanine. Like glycine, these amino acids are members of the elite club of “coding” amino acids that are strung together to make all the proteins of the body according to the DNA code. Human cells are unable to synthesize these amino acids, because they don't have a shikimate pathway, so they are called “essential.” We have to acquire them from our diet or rely on our gut microbes to use their shikimate pathway to synthesize them for us, their host. When our food sources or gut microbes are exposed to glyphosate, they become impaired in their ability to supply us with these crucial nutrients, and we can become deficient.
Many biologically active molecules are derived from the products of the shikimate pathway, including the neurotransmitters serotonin, melatonin, dopamine and epinephrine, thyroid hormone, the skin tanning agent melanin and the B vitamins niacin and riboflavin. Niacin and riboflavin are going to play a central role in the discussion below, which will ultimately lead to an explanation of why glyphosate might cause COVID-19 to be a much more severe disease than it would otherwise be!
2 Deuterium
It was only in December 2019 when I first became aware that deuterium poses a significant challenge to biological organisms. Fortuitously, at that time, a professor of pediatrics at UCLA, Prof. Laszlo Boros, had reached out to me due to a paper I had published that he found of interest. That paper, which I wrote together with Greg Nigh, a naturopath, had developed an argument for how sulfate deficiency could motivate a complex reaction cascade that takes place when the B vitamin cobalamin (B12) is deficient. We centered the paper on the idea that sulfate supports the maintenance of gelled water (so-called “structured water”) in the glycocalyx that coats all the blood vessels in the body. We argued for an important role for the enzyme endothelial nitric oxide synthase (eNOS), particularly in the red blood cells, to produce sulfate in response to sunlight, using cobalamin as a cofactor. We showed how sulfate deficiencies brought on by cobalamin deficiency could lead to a major adjustment of metabolic policy and ultimately explain most of the diverse symptoms of cobalamin deficiency4. I now believe that the gelled water, stabilized by sulfate, is also good at retaining deuterium, creating a deuterium trap that helps reduce the deuterium burden in the cells.
Laszlo opened my eyes for the first time to the idea that deuterium matters a lot in biology. What is deuterium? Deuterium is a heavy isotope of hydrogen, the smallest element in the periodic table. Hydrogen has just one proton and one electron; deuterium is the same except that it has, in addition, a neutron (uncharged particle that is about the same size as a proton). This makes it about twice as heavy as hydrogen, and also makes it behave differently, both biochemically and biophysically.
Hydrogen is by far the most common element in the body. Sixty two percent of the atoms in the body are hydrogen atoms. Most of these are in water molecules. Water (H2O) contains two hydrogens and one oxygen atom. Up to 60% of the body's total weight is water, but water molecules make up nearly 99% of the body's total molecule count. Deuterium is a natural element, and it shows up everywhere. In seawater, only about 155 out of every million hydrogen atoms is a deuterium atom instead. But because there are so many hydrogen atoms in the body, this amounts to a significant amount of deuterium – it is estimated to have six times as high a concentration in the blood as the mineral calcium.
Protons are hydrogen atoms without their electron, and deuterons are deuterium atoms without their electron. Mitochondria are organelles inside cells that are the “energy generating factory” of the cell. They run the citric acid cycle to process nutrients like sugar into carbon dioxide and water. The process involves pumping protons across the inner membrane of the mitochondrial wall to build up a voltage drop, and then invoking an “ATPase pump” to pump the protons back across the membrane, while simultaneously converting oxygen into water through oxidative phosphorylation.
Laszlo Boros made me keenly aware of the importance of deuterium depletion in the mitochondria. He was the lead author on a fascinating paper that explained how the cells “scrub” deuterium from nutrients that feed into the citric acid cycle in the mitochondria, and then selectively pump protons into the intermembrane space of the mitochondria while carefully avoiding pumping in deuterons5. Deuterons have a nasty effect when they get into the pump – a bit like getting sugar into the gas tank. They cause a hiccup in the pump that releases dangerous reactive oxygen species, and they can cause the pump to break altogether. This results in “mitochondrial dysfunction,” a common feature of a broad range of metabolic, oncological and neurological diseases.
Niacin and riboflavin are critical players in the maintenance of low deuterium in the mitochondria. There is a set of enzymes that convert niacin into nicotinamide adenine dinucleotide (NAD), and another set that convert riboflavin into flavin adenine dinucleotide (FAD). These two molecules (NAD and FAD) are essential for maintaining low deuterium in the mitochondria, and the science behind this is complex but fascinating.
Both NAD and FAD contain a double phosphate unit that hooks the two nucleotides together (see Figure 1). There are many enzymes that bind NAD or its phosphorylated form NADP, which has three phosphate anions. There is also a class of enzymes called flavoproteins that bind FAD, and use FAD as a holding station for protons and electrons being passed along to effect the chemical reaction that the enzyme specializes in. Often the protons and electrons originate from an NAD molecule that the enzyme also binds to. eNOS, the enzyme that was discussed at length in the paper Greg Nigh and I published that caught Laszlo's attention, is a flavoprotein that binds both FAD and NADP. It also binds heme, another molecule that biology makes great use of to help facilitate chemical reactions, and one that will be revisited later in this article. Heme is also of course the molecule that contains iron bound to oxygen to support oxygen transport by hemoglobin in red blood cells.
Many enzymes, and pretty much all flavoproteins, have a special skill to select hydrogen (H) over deuterium (D) when they perform their reaction. There is a term called a “deuterium kinetic isotope effect” (KIE) that captures the extent to which a given enzyme can favor hydrogen over deuterium. They achieve this unique skill by invoking a cosmic physics principle called “proton tunneling.” Essentially, if things are set up just so, protons can cheat in a chemical reaction, avoiding an energy barrier by metaphorically “tunneling through” a hill.
Deuterons are much worse at proton tunneling than protons, and this is how these enzymes are able to select for protons over deuterons when they synthesize their product. Importantly, these specialized enzymes often move hydrogen from or to an NAD(P) molecule, causing either NAD(P)H to become NAD(P)+ or vice versa. What is important to realize is that the “H” in NAD(P)H is almost never D, because the enzyme's reaction fails when D displaces H. And what is also important to realize is that the mitochondria are very adept at grabbing the H from either NADH or NADPH and adding it to the water pool that's collecting deuterium depleted water (DDW), and they often use flavoproteins to do this. DDW is being widely marketed on the Web as a therapeutic form of water, and it is often as expensive as a good bottle of wine.
3 Glyphosate and Flavoproteins
You may have realized from the above discussion that glyphosate can be predicted to deplete the supply of both NAD and FAD to exposed organisms, because it disrupts the synthesis of the precursors coming out of the shikimate pathway. This is clearly one way in which glyphosate might compromise the mitochondria's ability to maintain DDW.
However, it is plausible that glyphosate disrupts deuterium depletion in a much more direct way – by suppressing the activity of the enzymes involved in the proton tunneling reactions. Flavoproteins have a signature motif at the site where they bind FAD, and this same motif shows up where enzymes, even enzymes that are not flavoproteins, bind NAD. This motif, a characteristic highly conserved feature of a so-called Rossmann fold topology, has the pattern “GxGxxG.” Here, “G” stands for glycine and “x” stands for “wildcard” – meaning that any amino acid, including glycine, could go there. So this motif has at least three glycine residues binding phosphate that could logically be substituted by glyphosate, disrupting the protein's ability to bind either FAD or NAD(P). Mutations of the glycines in this motif cause the enzyme to lose enzyme activity6. Glyphosate has been shown experimentally to suppress several different enzymes that bind to either NAD, NADP or FAD, including succinate dehydrogenase7, the class of cytochrome P450 enzymes in the liver8, Cytochrome P450 reductase9, NADH dehydrogenase10 and glucose 6 phosphate dehydrogenase (G6PD)11. It also suppresses RuBisCo in plants, which is critical for photosynthesis and is the most common protein in the world12. All of these proteins have in common that they bind to phosphate-containing dinucleotides.
4 Heme Oxygenase in COVID-19
In a previous article in a recent issue of this journal, I presented the argument that glyphosate in biofuels could be a significant factor in inducing acute sensitivity to COVID- 19, leading to a downward spiral into a life-threatening situation13. Air pollution is a known risk factor for bad outcomes, yet a country like Taiwan with poor air quality in the big cities nonetheless has had only a handful of deaths from COVID-19. Taiwan uses much less glyphosate than the US and does not use any biofuels in its vehicles. I also talked about the unusual lung disease that is showing up in people who use e-cigarettes, which is symptomatically very similar to COVID-19. E-cigarettes make use of glycerol, a by-product of the biofuel industry, as the solvent for the nicotine, and it is likely also contaminated with glyphosate.
It is clear that some COVID-19 cases cascade into a hyperinflammatory state in the blood. This induces an acute thrombotic response, with blood clots appearing throughout the vasculature, eventually leading to multiple organ failure and death. A recent publication suggested that the pathology might be caused by an insufficient production of an enzyme called heme oxygenase 1 (HO-1)14. Normally, under conditions of extreme stress, an inflammatory response induces increased production of HO-1, and HO-1 converts heme (the molecule that carries oxygen in the hemoglobin in red blood cells) into biliverdin (a green pigment excreted in bile, and an oxidized derivative of bilirubin), releasing carbon monoxide (a signaling gas that helps tame the in ammation).
HO-1 usually operates in the endoplasmic reticulum, but, under stressful conditions, it moves into the mitochondria. Importantly, along with biliverdin production, it converts three molecules of oxygen into three molecules of water, while converting three molecules of NADPH into NADP+. In other words, it produces deuterium-depleted water in the mitochondria! The restoration of NADPH from NADP+, crucial for sustaining the reaction, depends on an enzyme that glyphosate is known to suppress, namely CYP reductase15.
Besides reducing the supply of NADPH and interfering with the ability to maintain NADPH in its active, reduced form, how else might glyphosate interfere with HO-1? A highly significant fact is that the enzyme contains two critical glycine residues – G139 and G143 { that bind electrostatically to heme and secure it in place to allow the reaction to take place. If either of these glycines is mutated to a different amino acid, the enzyme can no longer successfully carry out its reaction.
The title of a paper published in 2000 clearly states the consequences of replacing the first glycine residue with a different amino acid: “Replacement of the Distal Glycine 139 Transforms Human Heme Oxygenase-1 into a Peroxidase.”16 They replaced it with several different amino acids, but the one that caused the worst disruption was aspartate. Aspartate is a very good model for glyphosate, because, like glyphosate, it is considerably larger than glycine and negatively charged. Turning the molecule into a peroxidase would result in the release of redox-active ferryl iron (Fe(IV)) – a highly oxidized and very dangerous form of iron17. Worse than this, the reaction that converts heme to biliverdin is completely blocked, so the mitochondria receive no benefit from the production of deuterium depleted water.
This predicted effect is borne out in evidence from people who have a genetic mutation in G139. A paper describing a patient who picked up a bad copy of HO-1 from both parents is titled: “Mutating heme oxygenase-1 into a peroxidase causes a defect in bilirubin synthesis associated with microcytic anemia and severe hyperinflammation.”18 Severe hyperinflammation is perhaps the best descriptor for acute COVID-19 infections.
The toxic effects of the ferryl iron released by defective HO-1 is a direct path to ferroptosis. Ferroptosis is a newly identified form of regulated cell death resulting from iron- dependent accumulation of oxidized lipids that disrupt membrane function19. Interestingly, ferroptosis is linked to the loss of a sense of taste that occurs in some patients with COVID-1920. Highly oxidized iron also interacts with proteins in the coagulation cascade to induce the massive formation of blood clots leading to multiple organ failure. A paper published in the August, 2020 issue of the International Journal of Infectious Disease proposed specifically that excess release of toxic iron due to “altered iron homeostasis” could be the mechanism by which COVID-19 spirals downward into a coagulation cascade and multiple organ failure21.
Thus, the consequences of glyphosate perturbing HO-1 by substituting for G139 would be catastrophic, because there is a dangerous positive feedback loop where inflammation induces HO-1 which induces more inflammation. Normally, HO-1 resolves inflammation, but the opposite can be predicted to occur when glyphosate disrupts the protein through glycine substitution at the heme-binding site.
5 Conclusion
This article has been a whirlwind tour through many concepts in biology that are likely unfamiliar to most readers. But hopefully you can appreciate the science enough to realize that glyphosate can be predicted to disrupt the body's ability to fractionate deuterium and keep the deuterium levels in the mitochondria at a healthy state. I believe it is even possible that an overzealous inflammatory response is an indicator of deuterium dysbiosis. It is clear that upregulation of HO-1, which normally would resolve inflammation, also serendipitously supplies deuterium depleted water to the mitochondria. Thus the resolution of the inflammatory response coincides with the resolution of the deuterium excess problem in the mitochondria. But these processes get derailed by glyphosate, and this can have life-threatening consequences for someone who is infected with SARS CoV-2.
To help stay safe against COVID-19, it is important to take three simple steps: 1) Eat only certified organic food, 2) Stay away from interstate highways and heavily air-polluted inner cities, and 3) get out in the sunlight to help stimulate the production of sulfate in the skin.
References
- A Samsel & S Seneff. J Biol Phys Chem 2016; 16: 9-46.
- S Gunatilake et al. Int J Environ Res Public Health 2019; 16(15): 2734.
- S Eschenburg et al. Planta 2002; 216: 129-135.
- S Seneff & G Nigh. Water 2019; 11: 22-42.
- László G Boros et al. Medi Hyp 2016; 87: 69-74.
- CA Bottoms et al. Protein Sci 2002; 11(9): 2125-2137.
- N. Benachour & G-E Séralini. Chem Res Toxicol 2009; 22(1): 97-105.
- E Hetanen et al. Acta Pharmacol et Toxicol 1983; 53(2): 103-112.
- S Richard et al. Environ Health Perspect 2005; 113(6): 716-720.
- W Lu et al. Molec BioSyst 2013; 9: 522-530. Supplementary Table S2.
- D Cattani et al., Toxicology 2014; 329: 34-45.
- D Vivancos et al. Plant Physiol 2011; 157(1): 256-68.
- S Seneff. Masters of Health July 2020; pp. 76-83.
- PL Hooper. Cell Stress Chaperones 2020; 4: 1-4.
- Y Higashimoto et al. J Biol Chem 2005; 280(1): 729-37.
- Y Liu et al. JBC 2000; 275(44): 34501-34507.
- T Gáll et al. Int J Mol Sci 2019; 20: 3675.
- J Greil et al. Haematologica 2016; 101(11): e436e439.
- Y Suna et al. Biomedicine & Pharmacotherapy 2020; 127: 110108.
- T Osaki et al. J Oral Pathol Med 1996; 25: 38-43.
- M Edeas et al. Int J Infect Dis 2020; 97: 303-305.